What is gene transfer?
An individual gene carries the information that encodes the structure of a protein. Therefore, transfer of the
gene from one organism to another can enable the protein to be produced in an organisms where the gene had not
been initially present. The transfer can ultimately lead to the insertion of a gene from a plant or animal or bacteria
into a different plant, animal or bacterial species. However, in all cases two distinct steps are required to carry
out the transfer:
Step 1, isolation: Since genes occur as tiny fractions of the entire length of unwieldy chromosomes, the various
operations for utilizing a gene start by incorporating the gene into a smaller structure that is easier to isolate
and to handle. What is needed is a length of DNA just sufficient to encompass the gene.
Step 2, transfer: In order to complete the transfer, the DNA of the gene must be associated with additional DNA
elements that permit the ensemble to replicate in cells that are easy to grow (such as a bacteria). The ensemble
generally also includes the presence of some "markers" on the DNA can facilitate screening for its presence.
Various DNA constructions of this type have been developed to carry a gene from one type of cell to another and
such constructions are called "vectors".
How is isolation of a gene (step 1) achieved?
All gene transfers begin with the identification of the gene. Some genetic diseases were first identified by observing
a modified protein (as in the case of sickle cell anemia) and the gene was later identified on the basis of the
structure of the protein. More commonly at the present time, a genetic disease is recognized by clinical symptoms
and the gene is then identified through analysis of afflicted families. The identification of genes associated
with a disease will be increasingly facilitated as all of the genes in the human genome are identified in the completed
DNA sequence.
Once identified, the gene must be isolated in a suitable form and amplified to make it available in sufficient
quantities for subsequent steps. Isolating a gene from a large genome, such as the human genome with tens of thousands
of genes, requires particularly specific methods to find the gene of interest among such a large number of other
genes. Two approaches can be used for isolating a gene. One approach involves screening for a fragment of DNA that
contains the gene of interest using a gene bank within bacterial viruses called phage. In this case, once a phage
containing the gene is isolated, amplification can be achieved by growing the phage in sufficient quantities to
provide a source of the DNA for completing the transfer, as summarized below. The other approach involves isolating
and amplifying the gene in a single procedure directly from a preparation of DNA using specific probes in conjunction
with a powerful method called PCR (for polymerase chain reaction) in order to provide sufficient quantities.
PHAGE GENE BANK
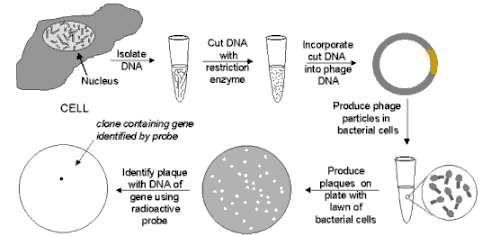
LEGEND: Phage Gene Bank: When bacteriophage are
grown on a continuous lawn of bacteria on a culture plate, small clear spots (called "plaques") will
appear where one virus has infected one cell and then, after reproducing, has infected the neighboring cells, with
the process repeating until a visible plaque is formed. If a complete sample of human DNA has been cut into small
pieces of DNA and inserted into the DNA of an appropriate phage vector, when the phage are plated on a bacterial
lawn, each plaque will contain the DNA of just one or two human genes. (Pieces of genes will also be present, so
following isolation in this way, the DNA must be tested to insure that it contains the entire gene.) Then, if a
suitable probe of the gene is available (for example a chemically synthesized strand of DNA whose composition is
determined -- using the genetic code -- from the amino acid sequence of the protein specified by the gene), the
probe can be added in radioactive form. It will hybridize to the DNA only of phage particles that carry the gene.
Then the plates are covered with photographic film and a spot will appear only where the radioactivity darkens
the film. In this way phage particles from the plaque can be isolated, grown in large quantities, and the DNA from
the phages can treated with an appropriate restriction enzyme to isolate the gene.
What is the basis for isolating a gene by PCR?
With PCR, a gene or any fragment of DNA can be amplified, by using two specific "primers" that bind to
the DNA on each side of the region to be amplified. The method works by repeating a cycle of reactions many times
that involves heating the DNA to separate the strands, lowering the temperature to bind the primers, and extending
the primers to synthesize a new strand of DNA. The extension step is carried out in the presence of a supply of
the 4 bases in the appropriate form and a special polymerase that is able to operate at relatively high temperature.
The number of copies of the gene defined by the two primers doubles with each reaction cycle. Typically about 30
cycles are used, so beginning with just one copy of the gene to be amplified, the number of copies at the end of
the 30 cycles would be 230 or about one billion.
How is transfer of the isolated gene (step 2) achieved?
In order for transfer to be carried out, the gene must be incorporated into an appropriated vector, one that is
designed to take into account the final destination of the gene, as well as the host in which the vector will be
grown. The vector is often a bacterial plasmid, but specific vectors exist for yeast and other cells used in the
cloning process. Bacterial plasmids are the generally used because of the rapidity of growth of bacteria and the
ease of handling. Plasmids are small circular molecules of DNA (usually containing about 100,000 base pairs) that
are capable of replicating freely within a bacterial cell, generally the common gut bacteria of humans, Escherichia coli. Their replication requires a specific DNA sequence called an "origin of replication"
and plasmids are generally constructed with several genes that can serve as selectable markers to test whether
the gene of interest has been correctly inserted and whether the bacterial cells have incorporated the plasmid.
In the example presented below the plasmid contains a gene for resistance to the antibiotic tetracycline plus a
gene for resistance to another antibiotic, ampicillin. By inserting the gene to be transferred into the plasmid
at a position that interrupts the ampicillin gene, the plasmid can no longer confer resistance to ampicillin. Therefore,
when the plasmid containing the new gene is added back to bacterial cells, only cells containing the plasmid will
grow on tetracycline. However, when these same cells are tested on ampicillin, they will be killed, so only tetracycline-resistant
cells that are ampicillin-sensitive will be retained for the further cloning steps.
BACTERIAL PLASMID USED AS A VECTOR
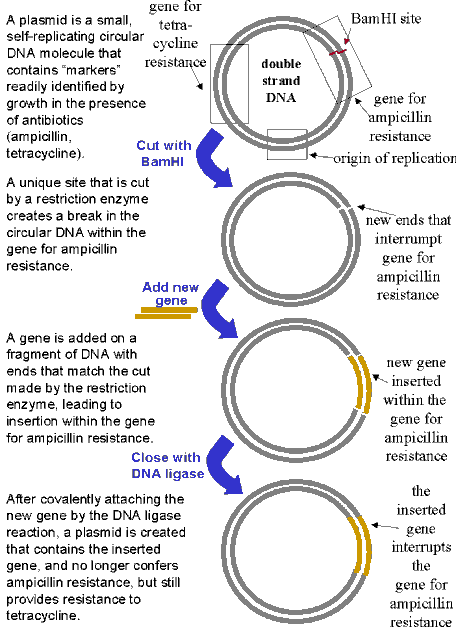
What specific tools are used to incorporate a gene into a plasmid?
The actual transfer of a gene into a vector is carried out with the aid of specialized enzymes, called restriction
enzymes, that cut the DNA only at certain specific sequences. In the example presented below, the enzyme BamHI
cuts DNA between the adjacent G's on both strands (indicated by the vertical arrows):

Restriction enzymes typically cut DNA at a sequence that is "palindromic," that is the sequence on one
strand is identical to the sequence on the other strand read in the opposite direction. As a result each strand
is left with identical "sticky ends" (....GATCC in this example) that can be used to attach an added
fragment of DNA (with the gene of interest) that also contains these sticky ends. When the DNA containing the new
gene is added, the sticky ends are stabilized in chemical bonds by the use of another enzyme called DNA ligase.
By choosing a restriction enzyme that cuts the DNA within the ampicillin gene, as shown in the figure for BamHI,
the capacity of the plasmid to confer resistance to ampicillin will be lost when the gene to be transferred is
inserted.
Depending on the objectives, the gene may be transferred to other vectors designed for specialized purposes. For
example, if the objective is production of the protein produced by the gene, a vector will be used that carries
a powerful promoter in front of the gene, to insure that the protein is expressed in large quantities. If the protein
is to used to transform mouse cells, a different type of vector is needed, as described in detail in the section
for introducing genes into mice. For transformation of human cells, the most commonly used vectors are viruses
that have been inactivated with respect to any harmful activities, but in which new genes have been added for delivery
to the target cells.
What are the specific applications of gene transfer?
Gene transfer refers to a range of modifications in the genetic make-up of an organism. The broad categories include:
- Genetic reprogramming of bacteria or other "production" organisms in order to obtain
proteins for industrial or medical purposes. Examples of this type of application include introducing the gene
for a human protein such as insulin that can be used to produce insulin from bacteria for medical purposes. While
bacteria are the organisms that are the most conveniently modified (and easy to grow rapidly in large quantities),
for certain applications it is preferable to use yeast cells, plant or animal cells in culture, or entire plants
or animals. Considerable success has been achieved with all types of cells in culture, and with entire plants.
In one striking example, human hemoglobin was produced in tobacco plants. For large animals, preliminary results
have been achieved in some cases for the production of proteins, for example production of small quantities of
human clotting factor IX in the milk of goats.
- Genetic reprogramming of plants for crop production. Methods have been developed that permit extensive genetic
modification of plants, including those that are now at the center of the controversy involving genetically-modified
crops that are opposed by some consumer and ecology groups. Genes can be conveniently transferred to plant cells
and production of complete plants from single plant cells is readily achieved. In this respect the cloning of plants
is much simpler than the cloning of animals. Crops that produce pesticides have been available for some time and
recently a yellow form of rice has been produced that synthesizes the precursor of vitamin A.
- Genetic reprogramming of mice for experimental
purposes. Among mammals, research
on mice has progressed to an advanced stage, permitting selected genes within the germ cells to be eliminated (gene
knock-out) or introduced (gene knock-in), thereby leading to the production of genetically-modified mice in which
all cells of the animal have been modified. Moreover, by combining gene transfer with the insertion of certain
DNA sequences (called promoters) that control the expression of genes, it has been possible to produce animals
in which the desired gene is expressed only in certain tissues. A striking application of these methods was the
recent report concerning production of "smart" mice with extra neurotransmitter receptors in their brains.
The mice are considered "smart" because they exhibit superior ability in learning and memory for various
behavioral tasks.
- Genetic reprogramming of humans. Compared to the achievements with mice, the status of genetic
modifications of humans is much more limited, for both technical and practical reasons (as well as obvious ethical
reasons). The modification of a gene in all the cells of a human being is far beyond current technical abilities
and ethical considerations also impose restrictions. To date, limited success has been achieved that involved introducing
a correct gene into certain cells of individuals with a deficiency in that gene due to a mutation. For example,
a rare immunological genetic disease (SCID) has been treated by introducing a gene into lymphocytes. In addition,
encouraging preliminary results were obtaining by introducing a gene for a coagulation factor into muscle cells
of hemophilic individuals.
Why is gene transfer so important?
Gene transfer permits genetic modifications to be accomplished that would be extremely laborious at best, but often
completely impossible to achieve, by classical genetic methods -- mutagenesis, many generations of mating, and
screening of mice to determine if only one gene had been affected. Therefore, where the goal is to develop an organism
with a specific genetic modification, gene transfer represents a method that provides a dramatic short cut compared
to classical methods.
How is a gene introduced into a complex organism, such as mouse?
Many of the methods currently used on animals were developed in mice, since mice are easy to handle and reproduce
rapidly. Mouse embryo-derived stem (ES) cells can be grown in culture and genes introduced with the appropriate
vectors. Stem cells have the capacity to differentiate into any of the specific types of cell (nerve, muscle, skin,
bone, liver, etc.) found in an adult organism. Although the genes are not always introduced correctly or efficiently
into all stem cells treated, clever methods have been developed that use special markers to test whether the gene
has been properly inserted. A "positive marker" allows only those cells to grow that have inserted the
new genetic construction and a "negative marker" kills cells that have inserted the genetic construction
incorrectly. Then the transformed cells can be inserted into an early embryo. The transformed cells will then give
rise to certain tissues and organs and in some cases the gene will be incorporated into sperm. When mice that produce
sperm containing the modified gene are mated with normal mice, mice that are heterozygous for the mutation in all
of their cells will be produced. One-quarter of the progeny of such heterozygous mice will be homozygous for the
transformed gene. The overall process thus involves several complicated steps, but it is routinely practiced in
many laboratories throughout the world. The various steps are examined in more detail below.
The initial phase of the methods involves the exchange of DNA sequences between the newly added sequences introduced
into an appropriate vector and sequences present in the ES cells. This process developed by Mario Capecchi in Utah
is called "gene-targeting." It takes advantage of a natural cellular phenomenon called homologous recombination
by which strands of nucleotides from an added gene align with matching sequences already present in the cell. When
the newly inserted gene finds its target, it will line up with it and replace it in a small fraction of the cells
treated. The process, summarized in the figure below, occurs even if the new gene carries inserted sequences that
turn off the original gene or modify its activity, since the larger gene will loop out, as depicted in the figure,
allowing the sequences at the extremes to find their homologous partners. When this process does occur, it will
also introduce the "positive" marker in the cell (for example resistance to neomysin) since the gene
for the resistance is used to interrupt the gene that is homologous to the targeted gene. Hence, only the transformed
cells will survive when grown in the presence of neomysin. A "negative" marker, for example the gene
for DTA, (the A fragment of diphtheria toxin that kills cells by interfering with protein synthesis) is also used,
since the gene will be eliminated in cells that correctly incorporate the interrupted gene in place of the target
gene by homologous recombination. However, in cells that have incorrectly incorporated the interrupted gene, homologous
recombination is not involved and the entire construct including the DTA gene will be inserted at random. These
latter cells will express the DTA gene and be killed by the toxin. Thus when cells are grown in the presence of
neomysin only those that have correctly inserted the interrupted gene will survive.
HOMOLOGOUS RECOMBINATION
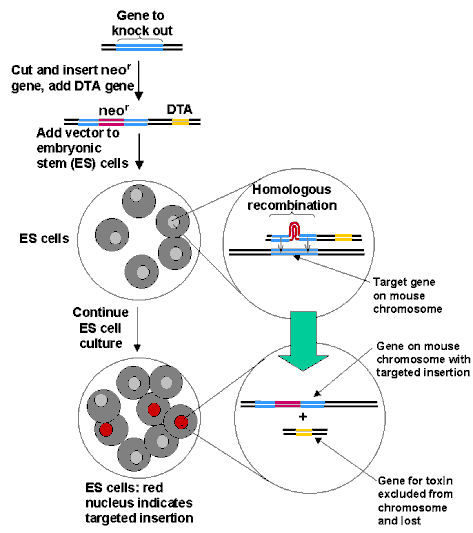
The correctly modified ES cells are then used to
produce chimeric mice, and ultimately mice with a specific gene knockout, as summarized in the figure below:
PRODUCTION OF CHIMERIC MICE FOR
GENE KNOCK-OUT
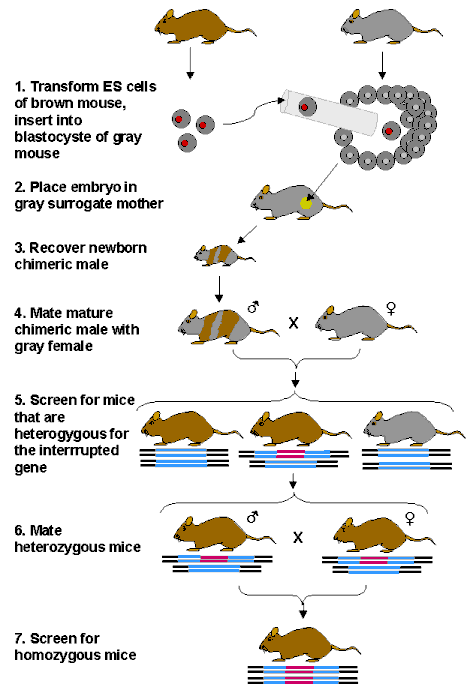
A powerful application of the method has been to
insert DNA sequences that correspond to a gene with a large interruption, so that when homozygous mice are produced
the gene of interest is effectively eliminated. Hundreds of different lines of mice with such a gene knock-out
have been produced in laboratories around the world and have provided numerous insights into the function of the
genes in question. Similar methods have been used to insert specific genes (gene knock-in).
Gene transfer methods can be applied to large animals, but each step is more laborious, much longer to carry out,
more expensive, and therefore difficult to maintain for a large enough number of animals to insure success. In
principle, once a genetically modified mouse or large animal is produced, exact copies could be reproduced by cloning,
that is taking the nucleus from a somatic cell and transferring it to an egg cell. However, although much excitement
was generated by the cloning of sheep, notably Dolly, and other animals subsequently, the methods for cloning are
extremely inefficient and unreliable, and as a result are not yet of practical use. Much more basic knowledge in
biology is required before such approaches can be considered well established.
Do the same principles apply to plants?
Genes can also be readily introduced into plants using methods similar to those employed for animals. Indeed, plants
possess a number of advantages that enabled gene transfer to be applied on a large scale for plants earlier than
for animals. Some special steps are needed, however, because of the cell wall of plants that makes the introduction
of foreign DNA more difficult. One common solution is the "gene gun" that literally shoots genes into
plant cells. However, once a genetically modified plant cell is produced, obtaining plants and seeds is relatively
straightforward. Compared to the difficulties of Dolly-type cloning of animals, cloning of plants is based on methods
that have been solidly established for decades. Plant cells are generally totipotent, that is any cell can be isolated
and when properly cultivated will grow into a full plant, complete with many genetically modified seeds. Therefore,
genetically modified plants can be produced more readily than genetically modified animals, especially mammals.
As a result the practical issues of genetically modified organisms concern almost exclusively plants that have
been modified plants to produce their own pesticides or yield improved nutrients, such a rice modified to produce
provitamin A.
|